Changing Lives with Gene Therapies

The process of bringing new drugs and products to market requires creativity, thinking outside the box, and the courage to fail numerous times before making a single discovery. This rings especially true now, as the industry faces the COVID-19 pandemic and doubts about vaccines and therapies getting to market in record time to improve human health. This article presents a deep dive into gene therapies, the path to those therapies from vaccine development in the 1940s and 1950s, and how the road to developing these has forever changed the landscape of modern medicine.
A look back at vaccine development illustrates the road that the industry has traveled. In the 1940s and 1950s, the poliovirus ravaged the world. In the 1940s, the paradigm for vaccine development was to isolate a live but weakened microorganism. The attenuated virus would then be administered to subjects to produce a low-grade, innocuous infection to confer long-standing immunity. Dr. Jonas Salk’s approach was unique, and he and colleagues developed noninfectious killed poliovirus (using formaldehyde) that maintained its antigenic properties.1 Drs. Albert Sabin and Hillary Koprowski also worked on the poliovirus in the 1950s, and they developed an live attenuated poliovirus vaccine, which was a trivalent oral vaccine that contained all three strains of the poliovirus.
The Path to Gene Therapies
We then fast forward to a time when researchers looked to viruses to potentially deliver corrected genes for genetic disorders (see Figure 1). Initially, many people thought this was an outlandish idea that would not come to fruition. However, in the late 1970s, Dr. Jude Samulski discovered how to clone the adeno-associated virus (AAV) during his doctoral studies, which helped pave the way for the use of gene therapies in many incurable diseases.2. In 1988, the goals of the Human Genome Project were first declared by the U.S. National Academy of Sciences. The project grew to become a collaborative, international program to map and understand all human genes.3 I was fortunate to be part of a research team working at the National Institutes of Health on the Human Genome Project to identify genes involved in brain development.4 Francis Collins, then-director of the National Human Genome Research Institute, stated after the publication of the majority of the genome in February 2001, “It’s a history book—a narrative of the journey of our species through time. It’s a shop manual, with an incredibly detailed blueprint for building every human cell. And it’s a transformative textbook of medicine, with insights that will give health care providers immense new powers to treat, prevent, and cure disease.”
Adenoviruses (AdVs) are nonenveloped, icosahedral DNA viruses with virion sizes ranging from 70 to 90 nm that belong to the diverse Adenoviridae family.5, 6 AdVs were first isolated from human adenoid tissues in 1953. AdVs usually cause nonsymptomatic respiratory tract infections in both humans and animals, but they can be life-threatening to immunocompromised individuals.7, 8 Moreover, the ability of AdVs to infect many cell types facilitated their exploitation as vectors for gene delivery to generate new tools for innovative treatments of important diseases, such as cancer and cardiovascular disorders.9, 10, 11, 12
AAV
AAV is a small, 25-nm nonenveloped virus with a linear single-stranded DNA genome that belongs to the Parvoviridae family.13, 14 To date, 13 distinct serotypes (AAV1–AAV13) exist, more than 100 variants have been isolated from human/nonhuman primates, and no diseases have been associated with AAV infections.14, 15, 16,17AAV serotypes have different transduction efficiencies for different tissues, which are dictated by their capsid sequence.16, 18 The transduction efficiencies of AAV serotypes have been harnessed to target a particular tissue or cell type to deliver gene therapies. The first AAV vector-based clinical trial was performed over 20 years ago; it used a cystic fibrosis transmembrane regulator (CTFR) transgene via a recombinant AAV vector (rAAV) in adult cystic fibrosis patients with mild lung disease.19 In 2012, the European Commission approved an rAAV1 vector encoding lipoprotein lipase as a therapy treatment for patients with this enzyme deficiency.20
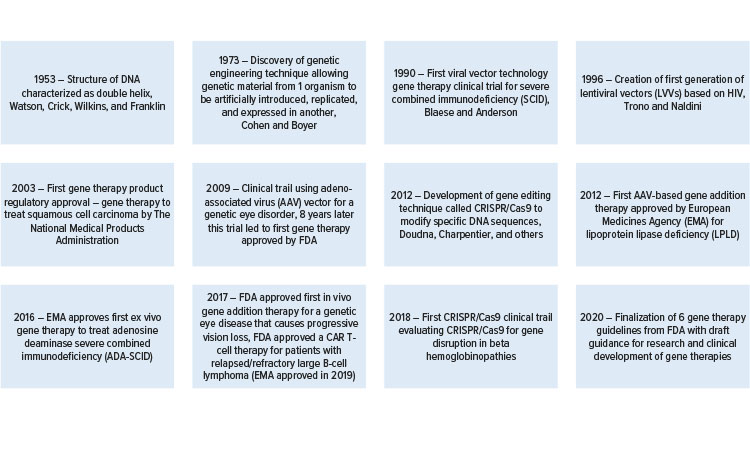
AAV is a preferred vector for gene therapy because of its lack of disease associated with wild-type virus, the ability to transduce nondividing cells, and long-term robust transgene expression. AAV vectors are the preferred delivery system for in vivo application because they deliver systemically via the vascular system, are nonpathogenic, and can infect a broad range of dividing and nondividing cells, and the various serotypes display differential tissue tropism.14, 21, 22 AAV vectors have essentially replaced the adenovirus for gene delivery with the initiation of a large number of clinical trials, with more than 100 trials either recruiting or active/not recruiting in the US.23 In contrast to the adenovirus vectors used in gene therapy studies, AAVs elicit a milder course of innate immune reactions, which is a key factor of a favorable safety profile and low toxicity.24
Genome Editing and CRISPR
Genome editing, a method used to precisely alter the DNA sequence of a cell, is broadly used as a research tool and is now being used to develop gene therapies. As progress in gene editing techniques continued, the CRISPR-Cas9 system (or CRISPR, which stands for clustered regular interspaced short palindromic repeats) emerged.
In 2011, Drs. Emmanuelle Charpentier and Jennifer A. Doudna were interested in a bacterial immune system that used an enzyme called Cas9 to “cut up” the genes of invading viruses. Charpentier published findings on how a pair of bacterial RNA molecules controlled this process.25 The scientists then developed a way to turn the viral defense system into a programmable gene-editing tool, and they synthesized a new molecule, the single guide RNA, which merges the key features of the two bacterial RNAs and directs Cas9 to cut a specific site in the DNA [26].
In 2020 Charpentier and Doudna won the 2020 Nobel Prize in Chemistry “for the development of a method for genome editing.”27 According to Fyodur Urnov, a gene-editing scientist at the University of California, Berkley, “The number of discoveries in biomedicine that have had the impact that Jennifer’s and Emmanuelle’s had can be counted on the fingers of one hand: recombinant DNA, PCR (polymerase chain reaction), DNA sequencing, and now CRISPR. We have never had a technology as powerful and versatile as genome editing with CRISPR.”
The CRISPR-Cas9 system is a powerful tool for genome editing because it is simple to use, demonstrates site-specific activity, and has limited off-target effects.28 The CRISPR-Cas9 system consists of the Cas9 nuclease that, when complexed with a short guide RNA, can be directed to create a double-stranded break in a wide variety of DNA sequences in both eukaryotic and prokaryotic genomes.26, 27 CRISPR-Cas9 systems are being applied to create new cell therapies, either through ex vivo editing of cells followed by transplantation of engineered cells into a patient or through in vivo editing of a patient’s cells by delivering the CRISPR-Cas9 system using viral vectors (AAV) or nanoparticles.28, 29, 30, 31, 32, 33, 34
The ability to attack genetic disorders at their root cause will forever change our world and provide hope where there was none.
The CRISPR-Cas9 system has been adapted from multiple bacterial species—including Streptococcus pyogenes, Streptococcus thermophilus, and Neisseria meningitidis—to efficiently generate targeted gene modifications in human cells and consists of a Cas9 nuclease that is co-expressed with a single guide RNA molecule.26, 28, 35, 36, 37 Cas9 forms a complex with the 3’ end of the single guide RNA, and the protein-RNA pair recognizes its genomic target by complementary base pairing between the 5’ end of the single guide RNA sequence and a predefined 20-base pair DNA sequence, known as the protospacer.38 By simply exchanging the 20-base pair recognition sequence of the expressed single guide RNA, the Cas9 nuclease can be directed to new genomic targets.39
Case Example
With an understanding of how viral vectors and CRISPR have changed the landscape of modern medicine, let’s review an example of using both of these technologies to provide a cure to a fatal genetic disease, Duchenne muscular dystrophy (DMD).
DMD is a rare, X-linked, fatal, degenerative neuromuscular disease that is estimated to affect 1 in 5,000 newborn boys worldwide.40, 41, 42 DMD is caused by mutations in a single gene, the DMD gene, that result in the lack of a functional dystrophin protein.43 Dystrophin links the F-actin in the cytoskeleton with beta-dystroglycan and the extracellular matrix through its N- and C-terminal domains, respectively.44 Mutations causing the premature truncation of dystrophin translation result in nonfunctional and unstable dystrophin proteins that are typically undetectable with standard diagnostic techniques.45 Dystrophin is a critical component of the dystrophin-associated protein complex in muscle cells. It is not only essential for the maintenance of muscle fiber stiffness but also for protection against mechanical stress during muscle contraction.46, 47, 48, 49
Given the essential role of dystrophin in protecting skeletal and cardiac muscle cells from mechanical stress, patients with DMD experience loss of critical functions resulting from progressive muscle tissue degeneration with motor symptoms.50, 51 In young males diagnosed with DMD, motor delays are followed by a functional decline, resulting in the loss of ambulation in their early teen years, wheelchair dependency, and an inability to perform daily activities, such as feeding. 23 Additionally, loss of diaphragm function can result in increasing respiratory impairment, cardiac dysfunction, and ventilator-assisted breathing in their teens and twenties, with disease progression leading to cardiomyopathy, respiratory failure, and premature death.52 There is no cure for DMD, and although palliative treatments are available (essentially, physiotherapy, assisted ventilation, and glucocorticoids), most patients have no satisfactory symptomatic or disease-modifying treatments.42
Over 7,000 different mutations have been reported in DMD patients.53 The commonality of these mutations is a nonfunctional dystrophin; however, many DMD patients can unexpectedly produce trace amounts of Becker muscular dystrophy (BMD)-type dystrophin.54 The deletion of exon 45 is one of the most common deletions found in DMD patients, whereas deletion of exons 44 and 45 is generally associated with a less progressive allelic form of muscular dystrophy, known as BMD.43 Therefore, if exon 44 could be bypassed in the pre-messenger RNA (mRNA) transcripts of these DMD patients, this could restore the reading frame and enable the production of a partially functional BMD-like dystrophin.44 Deletions of exons 44 and 45 are associated with very mild Becker phenotypes and have even been found in asymptomatic individuals.55 Patients with a deletion bordering exon 44 skip exon 44 spontaneously, although at very low levels. This results in slightly increased levels of dystrophin when compared with DMD patients carrying other deletions and most likely underlie the less severe disease progression observed in these patients compared with DMD patients with other deletions.43, 56, 57 Gene therapy for DMD should address the underlying genetic cause of the disease by specifically restoring functional dystrophin to key tissue targets in skeletal muscle, including the diaphragm and the heart, by introducing a functional gene to compensate for the mutated or absent dystrophin gene.
Novel Gene Therapy Approaches
Two companies using AAV viral vectors for the treatment of DMD are Astellas Gene Therapies (formerly Audentes Therapeutics) and Regenxbio. Astellas Gene Therapies uses the AAV vector approach, encoding a modified U7 small nuclear RNA (snRNA) to carry an antisense sequence to induce cells to skip over faulty sections of the genetic code in the dystrophin gene to restore productive levels of functional dystrophin protein. 58 Astella Gene Therapies is collaborating with Nationwide Children’s Hospital to conduct a first-in-human clinical trial with this gene therapy in DMD patients who have a duplication of exon 2 in the DMD gene.58 Currently, both preclinical and ongoing clinical studies support future AAV-U7 snRNA-based exon-skipping therapies to treat DMD. Furthermore, AT702, an AAV-antisense therapy that induces exon 2 skipping to treat DMD patients with duplications in exon 2 and mutations in exons 1–5 of the dystrophin gene, is being developed.58 Two additional products are being developed by Astellas Gene Therapies, AT751 and AT753, which are exon-skipping therapies indicated in the treatment of DMD patients with genotypes amenable to exon 51 and exon 53 skipping.58 The same vector backbone for AT702 is used for both AT751 and AT753, which allows for accelerated development for use in the clinic. Across these three products or programs, 25% of DMD patients can be treated, with future candidate therapies targeting up to 80% of DMD patients.58
The Regenxbio approach is different from that of Astellas Gene Therapies, but it also uses an AAV vector to deliver therapy to muscle cells. RGX-202 uses a proprietary novel AAV (NAV) vector of the serotype 8 capsid (AAV8) that encodes microdystrophin to be delivered to muscle cells.59 The NAV Technology Platform is composed of exclusive rights to AAV7, AAV8, AAV9, and AAVrh10 and more than 100 novel AAV vectors, with over 100 patents and patent applications worldwide covering NAV vectors.60 There are numerous benefits to using the NAV vectors compared to early generation AAV vectors, including broad application across multiple disease states, the lower likelihood of triggering an immune response, improved gene expression with long-lasting treatment in a smaller dose, and a less-complicated manufacturing process.60 RGX-202 is composed of a unique C-terminal domain for improved function, a muscle-specific promoter (Spc5-12) to direct expression of microdystrophin in skeletal and heart muscles, and additional features to reduce immunogenicity and improve gene expression.59 RGX-202 is a single administration therapy, and the preclinical phase of development is in progress, with plans for first-in-human clinical trials in mid-2021.
Several methods can be used to deliver DNA or RNA to cells in our body and can be modified to deliver CRISPR components. The methods can be divided into two distinct categories: viral and nonviral. One nonviral method utilizes lipid nanoparticles (LPNs) and mRNA. Advantages of LPNs are expansion beyond delivery to the liver, increased potency, and improved tolerability. In the LPN platform, an encapsulated mRNA encoding Cas9 and a guide RNA, and, if necessary, a donor DNA template, are used to deliver these components to the liver. CRISPR Therapeutics has partnered with the Massachusetts Institute of Technology to develop drug therapies using LPN technology and CRISPR to target the liver.60, 61 The advantages of using mRNA with CRISPR for drug products are tissue specificity, expression duration control, and increased potency. Additionally, CRISPR Therapeutics partnered with CureVac for mRNA/CRISPR programs to target liver alignments.61
Viral vectors can target numerous organs to deliver DNA encoding Cas9 with guide RNAs into specific tissues in the body.61 Examples of organ systems being targeted for viral vector/CRISPR technology products include muscle, lung, and the central nervous system. AAV vectors are the primary vector being paired with CRISPR technology. Advantages of AAV as a viral vector for in vivo delivery include reduction of immunogenicity, self-inactivation, and improved tissue specificity.
As mentioned previously, with the numerous mutations that can lead to DMD, Vertex Pharmaceutical/CRISPR Therapeutics have several different gene-editing programs that target the many disease-causing mutations. Vertex Pharmaceuticals is partnering with CRISPR Therapeutics to combine an AAV vector with CRISPR technology to treat DMD.61, 62 This therapy uses an AAV vector to deliver DNA encoding Cas9 to guide RNA into specific tissues in the body, such as muscle tissue, to produce a gene therapy for DMD.61, 62 To treat DMD, Vertex Pharmaceuticals/CRISPR Therapeutics uses CRISPR gene-editing technology and AAV9 virus to restore dystrophin protein expression by reframing the mutated DMD gene.62
Conclusions
The genetic cause of DMD is known, but a treatment for the disorder did not exist until gene editing became a possibility, as is the case with numerous genetic disorders. The ability to attack genetic disorders at their root cause will forever change our world and provide hope where there was none. We live in an exciting time—a time where we can get therapies to people who had no way of reversing their genetic disorders and who now have the opportunity to undergo treatments to help control the different presentations of their diseases. I believe we will witness the successful curing of a variety of human diseases, both genetic and acquired.